Article of the Month -
September 2014
|
GRAV-D: Using Aerogravity to Produce a Refined
Vertical Datum
Daniel ROMAN & Xiaopeng LI, United States
1) This paper focuses upon
the aerogravity science necessary to support the production of a
cm-level accurate geoid height model. The background is the Gravity for
the Redefinition of the American Vertical Datum (GRAV-D) Project in 2008
with a goal of developing a new vertical datum realized through a
regional (continental scale) geoid height model. The paper was
presented at the 2014 FIG Congress in Kuala Lumpur, Malaysia.
SUMMARY
The U.S. National Geodetic Survey instituted the
Gravity for the Redefinition of the American Vertical Datum (GRAV-D)
Project in 2008 . This geoid model will serve as the realization of a
new vertical height system in the U.S.A., replacing NAVD 88.
Collaboration with Canada, Mexico and other countries in North America,
Central America and the Caribbean will ensure that this model can serve
as a regional vertical datum, which can be readily linked to a future
World Height System. In order to produce such a model, significant (3-8
mGal) biases that exist in many of the 1400 different terrestrial
gravity surveys over the U.S.A. must be detected and mitigated.
Furthermore, 10-100 km wide near-shore gaps in oceanic gravity surveys
needed to be surveyed. Satellite models do not have sufficient
resolution to do either of these tasks. Hence, aerogravity profiles were
collected to enhance the satellite gravity field model for such uses.
However, in order to use the aerogravity data, trackwise biases needed
to be first corrected. A simplified approach was taken to determine and
remove biases in the aerogravity profiles using a reference model
determined by blending EGM2008 with GOCO03S. A comparison between
aerogravity and the modified reference model off the Coast of the
Northeastern U.S. highlighted areas of systematic difference at the +/-
3 mGal level with lateral extents of about 100 km. These features would
translate into an equivalent 5-10 cm of systematic error in a geoid
model and indicate possible errors in the surface gravity data used in
EGM2008. A similar analysis over the Great Lakes region demonstrated +/-
10 mGal biases with the NGS surface gravity data and clearly marked
which surface gravity profiles need to be addressed. More sophisticated
techniques will be developed for this process in the future. The intent
though is that aerogravity will be used to detect and mitigate the NGS
surface survey data, which largely lack metadata that might otherwise
eliminate these errors. In this manner then, the satellite, airborne,
and terrestrial data will made consistent so as to produce seamless
gravity field model for accurate and precise vertical control.
1. INTRODUCTION
1.1 Background
The National Geodetic Survey (NGS) is responsible for
maintaining access to the National Spatial Reference System (NSRS)
within the United States. The two principal aspects of the NSRS of
importance to surveyors are the North American Datum of 1983 (NAD 83)
and the North American Vertical Datum of 1988 (NAVD 88). It has been
well established by previous studies (Snay and Soler 2000, Smith et al.
2013a) that both of these official datums have significant (meter level)
systematic inaccuracies. Although both datums demonstrate considerable
internal consistency, significant problems with absolute accuracy
require that these datums be replaced. This is particularly true for
NAVD 88 where 30-50 cm regional features are detected and the difference
between the zero elevation surface of the datum and the best
satellite-based estimates of the geoid is over a meter in the Pacific
Northwest. Both will be replaced in 2022 by new geometric and
geophysical datums as is outlined in the NGS Ten-Year Strategic Plan
(NGS 2013). This paper will focus upon the aerogravity science necessary
to support the replacement of NAVD 88 with a new vertical datum realized
through a regional (continental scale) geoid height model.
Such models are regularly developed from global
models that are refined with regional surface gravity data. Essentially,
the local gravity field enhances the global model to produce a regional
model of suitable quality. Any systematic errors in the surface gravity
data will be removed first to achieve the desired cm-level of accuracy
as given in the NGS Ten-Year Plan.
After 2022, the vertical datum will be realized by a
combination of GNSS measurement and a geoid height model. Once
horizontal coordinates are determined through GNSS technology, the geoid
height at that location will be interpolated from the gridded geoid
height model, and a simple linear formula will be applied to derive the
orthometric height. Since the expectation is that the GNSS-derived
geometric coordinates will be cm-level accurate, then the geoid height
model must be of a similar accuracy.
1.2 Paper Organization
This paper is focused on the use of aerogravity data
to search for systematic errors in the surface gravity data and to
evaluate their potential impact if not removed. Aerogravity profiles are
available from the NGS website for the Gravity for the Redefinition of
the American Vertical Datum (GRAV-D) Project (http://www.ngs.noaa.gov/GRAV-D/).
These data were minimally filtered so as to maximize recovery of the
gravity signal amplitude. Small biases with respect to satellite gravity
fields (generally < 2 mGal) and other noise remain and are left to the
discretion of the user to remove depending on their requirements. For
geoid modeling, it is helpful to remove these biases from the
aerogravity data to produce a more coherent recovery of the gravity
field. Such a consistent field better reveals geophysical signals
expressed across multiple profiles. The aerogravity data, overflying
all, can quality check the surface gravity field, comprised of data
sourced from terrestrial gravity measurements, shipborne surveys, and
gravity determined from satellite altimeters, and can serve to unify
them into a seamless whole.
The next subsection provides more background on the
GRAV-D Project. Section 2 focuses on the aerogravity that has been
collected as a part of GRAV-D. It presents brief subsections on how
missions were planned and processed. Section 3 analyzes data over the
Northeast U.S. to demonstrate the removal of biases from the aerogravity
profiles, and how that aids in determining defects in the existing
reference field models. The aerogravity signal is also downward
continued to the surface for a direct comparison to the existing point
surface gravity data held by NGS. This last step is most useful for two
reasons. Firstly, the downward continued airborne data can be used in
place of the surface gravity data for geoid modeling, at least for
selected spectral bands where the airborne data is superior (Smith et
al., 2013b). Secondly, it identifies potential biases in surface gravity
surveys which must be corrected for the resulting geoid model to meet
the desired cm-level accuracy. It is this second use which is discussed
later in the paper. The last section provides for an outlook and details
some of the future work required to meet the 2022 deadline.
1.3 Why GRAV-D?
The Gravity-Lidar Study of 2006 (GLS06) (Roman 2007)
collected aerogravity over the northern Gulf of Mexico over Alabama and
Mississippi. Gravity grids were generated from the aerogravity and
surface gravity data held in the NGS gravity database. A prominent
disagreement with 3-5 mGal features existed between the airborne and
surface gravity data in the region (about 150 km x 250 km) west of
Mobile Bay. Three East-West and twelve North-South aerogravity profiles
crossing the region all contained signal that differed systematically
from what was seen in the terrestrial data. A 2008 surface gravity
survey (Roman et al. 2010) over the same region confirmed this
difference, and definitively put the source of the discrepancy on the
historic terrestrial gravity data. Terrestrial data were collected at 10
km intervals using LaCoste-Romberg relative meters tied to multiple
absolute gravity sites determined from a Micro-g LaCoste-Romberg FG-5
absolute gravimeter. The new surface gravity data agreed with the
aerogravity, and both showed the same systematic difference with the
gravity data from the NGS database. The effect of this 3-5 mGal
systematic difference produced 10 cm of inaccuracy in the geoid model
for that region. With the requirement for a cm-level accurate geoid
model, the existing gravity measurements in the database are
insufficient, at least for this region. This problem in the surface data
would not have been identified without the airborne data.
Saleh et al. (2013) demonstrated that significant
(3-8 mGals) biases exist in hundreds of the 1400 different terrestrial
surveys that comprise the two million gravity points across the U.S.A.
These biases would make it impossible to derive a cm-level accurate
geoid model; thus the data must be "cleaned" somehow to remove these
biases.
The intent of this project is to use the aerogravity
to bridge the spectral gap between satellite and surface gravity data.
Spherical Harmonic Models (SHM’s) are used to represent the global
gravity field. Satellite data dominate the longest wavelengths (λ) at
lower degree harmonics in the SHM’s, which is where most of the power is
located. In Figure 1, the blue line shows the power by degree harmonic.
The variance (power) is higher to the left and falls off to the right.
The lower harmonics to the left correlate to larger features and longer
wavelengths, which means that satellite data are more sensitive to
larger features in the gravity field.
The length of the aerogravity surveys (generally 500
km long profiles) ensures that they contain signal at wavelengths that
are also observed by various satellite gravity missions, such as GRACE
(Drinkwater et al. 2007) and GOCE (Pail et al. 2011). Since the
aerogravity data are measured from a moving platform at higher altitude
(details below), it does not contain as much of the short wavelength
signal. The shortest wavelength signal, which extends farthest to the
right in Figure 1, is where larger degree harmonics correlate to smaller
scale features with less power. The red boxes in Figure 1 highlight the
portions of the power spectra where the aerogravity overlaps with both
the satellite observed gravity field and surface gravity data.
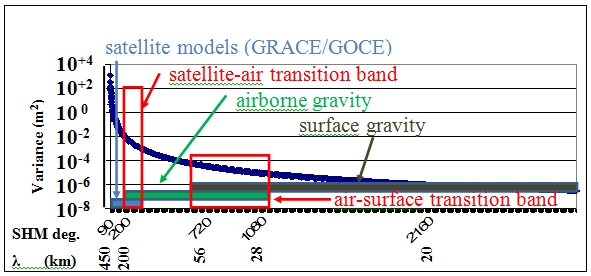
Figure 1 Power Spectrum plot of
gravity field (blue line). Most power is at longest wavelengths (λ) at
left on the lowest degree harmonics, where satellite (light blue bar)
data dominate. Surface data (brown bar) contain the shortest to the
right. Aerogravity (green bar) overlaps both parts of spectrum (red
boxes).
The satellite model will serve to unify regional
models by providing long wavelength consistency. Any inconsistencies in
the long wavelengths of the aerogravity will be ignored in favor of the
signal from satellite data. Ideally, the satellite model is augmented by
the aerogravity to produce a combined reference model independent of
surface gravity data in order to evaluate the surface gravity data. The
surface data will then be normalized by removal of biases and long to
intermediate signal trends. All data would then be consistent and could
be joined into a seamless gravity field model useful for defining a
vertical reference system that is both accurate and as precisely
repeatable as currently leveling methods. Hence, the GRAV-D Project
becomes a necessary component for replacing NAVD 88 in 2022.
2. GRAV-D AEROGRAVITY SURVEY
The GRAV-D Project has three components with the
airborne gravity collection campaign being the most recognized. See
Smith (2007) for other details about GRAV-D. The aerogravity campaign is
intended to collect direct observations of the gravity field from coast
to coast with a uniform coverage using consistent techniques.
2.1 Flight Planning
The GRAV-D airborne gravity campaign is designed to
span the entire continental U.S. and extend about 150 km into both
Canada and Mexico. This overlap provides sufficient coverage to
ultimately blend NGS geoid products with those of neighboring countries.
Coastal surveys extend beyond the shelf break to ensure collection in
the deep water regions where the gravity field is determined from
satellite altimetry data is deemed to be reliable (Sandwell, 1990).
Models of sea surface topography are better understood in the deeper
water beyond the shelf break. Thus the airborne gravity provides a
bridge between terrestrial gravity and deep-ocean altimetric data. The
GRAV-D Project will also span Alaska, the Aleutian Islands, Hawaii,
Puerto Rico, the U.S. Virgin Islands and the U.S. Pacific Island
territories of Guam, the Commonwealth of the Northern Marianas Islands,
and American Samoa.
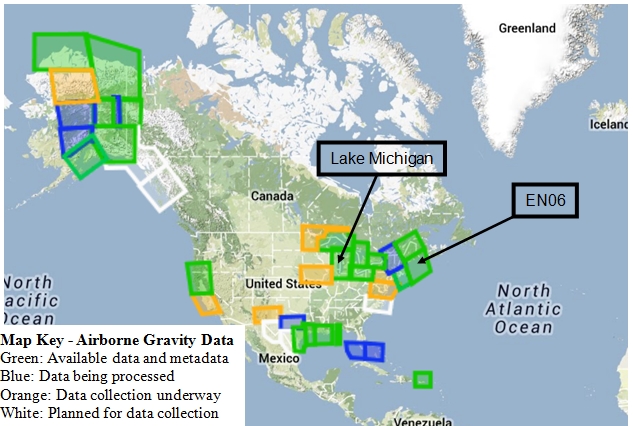
Figure 2 Extents of GRAV-D
Collections as of 11 February 2014. Inset box in lower left gives legend
on status of blocks. EN06 block in NE U.S.A. (Maine) and Lake Michigan
are discussed further below.
The GRAV-D Project has completed 35% of the U.S.
regions and is well on track for completion before 2022. Figure 2
highlights the status as of 11 February 2014. For up to date
information, see http://www.ngs.noaa.gov/GRAV-D/data_products.shtml. A
typical block is planned to be flown at 6.1 km (20,000 ft), with data
lines spaced 10 km apart, and flown at about 220 knots (~407 km/h. GPS,
IMU, and gravity meter readings are obtained and processed into a Level
1 product available for download from the GRAV-D website. A general
description of how these products are developed is provided in the next
section.
2.2 Data Collection and Processing
What is the waveband of reliability for the airborne
gravity data? The upper limit is determined by the shortest dimensions
of the survey block. Most GRAV-D surveys are rectangular and 400 km by
500 km in size to overlap the shortest wavebands of GRACE (300-400 km)
and GOCE (100-200 km) satellite gravity data. The shortest wavelength in
the airborne gravity is defined by sampling theory to be twice the
sampling interval, which in the cross track direction is twice the data
line spacing, or 20 km at full resolution or 10 km at half-resolution.
Data resolution along track is somewhat shorter than this, and is
determined by a combination of flight altitude (Childers et al 1999) and
the amount of along-track low-pass filtering used. At 6.1 km altitude,
gravity features below roughly 6 km in width (i.e. wavelengths below 12
km) are unlikely to be resolved. The along-track low pass filtering also
reduces the resolution of the signal, as a function of aircraft velocity
and filtering length. Data are measured at a 1 second rate which
provides a measurement every 113 meters (at the nominal 220 knots
velocity). A 120s time-domain Gaussian low pass filter is applied
sequentially three times, and based upon the nominal speed of the
aircraft, the filter is likely to suppress wavelengths below ~13 km.
Thus there is more spectral information in the direction of the data
lines (13 km minimum) than in the cross line direction (20 km minimum).
Hence for data collected at 6.1 km altitude, the gravity data exist
within the spectral band of 20 km to 400 km although the satellite data
are deemed to be more reliable in the long (300-400 km) wavelengths. At
10 km altitude, the waveband signal is essentially the same as the 6.1
km data because it is determined by the data line spacing and the survey
block dimensions, although the higher altitude attenuates signal
amplitude and results in greater noise in the downward-continued
product.
Further detailed information about the airborne
gravity data collection and processing for all blocks is available
online at the GRAV-D webpage along with the data at
http://www.ngs.noaa.gov/GRAV-D/. Also available at the website is
documentation for the aerogravity data collection process and a survey
report for each survey block.
3. ANALYSIS
The NGS Geoid Team is the primary customer for the
aerogravity data and is responsible for developing the required cm-level
accurate geoid height model for 2022. Systematic errors are first
removed or reduced in individual aerogravity profiles to better
determine the geophysical signal present as a Level 2 product. Then the
aerogravity may be used to assess the surface gravity data from the NGS
database.
The next two subsections cover both these aspects. A
simplified approach to bias removal in individual aerogravity profiles
is provided over the Northeastern U.S. (Maine). Blocks EN06, EN07 and
EN09 are used and also highlight the potential errors in the surface
gravity data used in EGM2008. These were flown in 2012 and processed in
2013. To demonstrate how aerogravity can then be used to evaluate
surface gravity data, the EN03 survey is used over Lake Michigan in the
Great Lakes region where significant surface gravity data problems are
known to exist. Block EN03 was collected and flown in 2013.
3.1 Northeastern U.S.A.
Figure 2 shows the location of a block of data (EN06)
spanning coastal Maine. The nearby green blocks are EN07 and EN09. The
intent of this subsection is to highlight how the aerogravity can be
compared against a global gravity field model that already contains
short wavelength signal derived from surface gravity data. The
supposition here is that systematic errors from surface gravity data
used in the global model contain biases and trends that will be detected
by the aerogravity. These biases and trends will show up as systematic
differences that will be highlighted in a plot of the differences
between the aerogravity and the SHM containing the surface gravity data.
An SHM was derived from the GOCO03S (Mayer-Gürr 2012) in combination
with the terrestrial grid for EGM2008 (Pavlis et al. 2012). This
improved reference model incorporates more GOCE signal while retaining
the short wavelength signal from the surface gravity used to build
EGM2008. Using this SHM, gravity disturbances were predicted at the
aerogravity observation points to form residuals.
Both the SHM and the aerogravity contain signal
between 20-400 km wavelengths. The aerogravity data were also tied to
ground control when the aircraft was launched and landed. So ideally
there should also be no long wavelength differences. Signal below 20 km
would have little power and would be negligible. Hence these residuals
should be near zero if the signal detected by the aerogravity were
consistent with both the existing surface gravity data used in EGM2008
and that from the GOCO03S satellite data.
Figure 3a highlights the residuals in grid EN06. Note
that many profiles show evidence of an unremoved bias or some systematic
effect ("trackiness"). The averages these residual profiles were used to
determine biases (Figure 3b), which were removed from the original
residual profiles. This residual profiles with the means removed are
shown for Blocks EN06, EN07, and EN09 (Figure 3c). Clear features are
seen that correlate between tracks indicating that there are real
geophysical signal differences between what is detected by the
aerogravity and what is indicated by the surface gravity data inherent
in EGM2008. The magnitude of these features is in the range of 3-5 mGals
but they correlate across a hundred or more kilometers.
This is a near shore region and so a number of
sources constitute the EGM2008 surface data. Data offshore were derived
from satellite altimeters. This data tends to break down in the near
shore environment and in backwater areas (Sandwell, 1990). Significant
negative and positive features can be seen to extend along the shoreline
and in bay areas in Figure 3.c. Onshore, data derived from terrestrial
gravity surveys, which were corrected for elevation and other
parameters. The SW-NE trending highs and lows correspond to features in
the terrain, which could indicate problems with the gravity observations
or the terrain models.
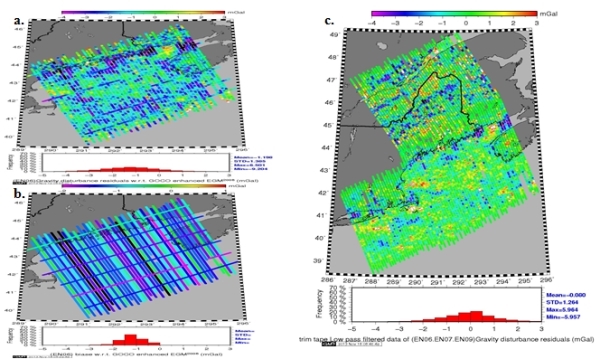
Figure 3 a. Residual gravity
disturbances (EN06 – SHM). b. Biases detected in EN06 profiles. c. EN06,
EN07, and EN09 residual gravity disturbances with biases removed.
Remaining features highlight differences between aerogravity and surface
gravity from the reference SHM. Residual features with lateral extents
across multiple profiles indicate real signal not just noise.
Additionally, the boundaries between the three
surveys are not strongly in evidence. Each of the surveys was planned
with some overlap to ensure continuous coverage and processed using the
same techniques. As a result, no systematic features exist between
survey blocks, and the combined model of all three presents a consistent
treatment of the residual gravity disturbances throughout the region
(i.e., there are no biases between the survey blocks).
There are likely some problems with determining
biases using this approach. If, as shown in Figure 3c., there are
systematic differences between the aerogravity signal and the surface
data, then this shorter wavelength signal slightly alters the overall
average resulting in an incorrectly determined bias value. However, the
above procedure removes these potential biases to a first order. Further
refinements will be required to better remove biases of the aerogravity
with respect to only the satellite signal at the longest wavelengths.
However, the fact that the differences exist between the aerogravity and
the existing surface data indicates that the aerogravity has discovered
some potential systematic errors in the surface gravity that may
preclude a cm-level accurate solution. Surface gravity surveys will next
be compared against aerogravity to determine the magnitude of the biases
in the terrestrial surveys.
3.2 Lake Michigan
It is desirable to see if any of the surface gravity
data held by NGS contain biases or trends that would impact geoid
modeling. In the previous subsection, it was shown that surface gravity
commonly used in developing global SHM had systematic differences that
may represent biases in that data. To develop a regional geoid of
sufficient accuracy, it is desirable to detect any such errors in the
NGS surface gravity data using the aerogravity. Satellite models will
likely pick up these differences as well but will not sufficiently
resolve the biases for individual surface gravity surveys.
Aerogravity data for block EN03 were treated in a
similar manner as above. The next step in the process is to harmonically
capture this signal using Least Squares Collocation (LSC) in order to
make the data regular for capture into a SHM. Since the SHM is a global
function, the aerogravity are used to update the local region of the
block survey, while retaining the original signal outside of the region.
The residual gravity highlights the difference between what the
aerogravity sensed and what was indicated by the reference model at
scales between 20-400 km. By modeling and incorporating these residuals
into an updated SHM, the local gravity field will reflect what was
sensed by the aerogravity and not was previously based on the surface
gravity data in EGM2008. As the GRAV-D Project progresses, more blocks
will be available to build into a larger region. In Figure 3 above,
three blocks were assembled. There are more than five blocks available
for the Great Lakes.
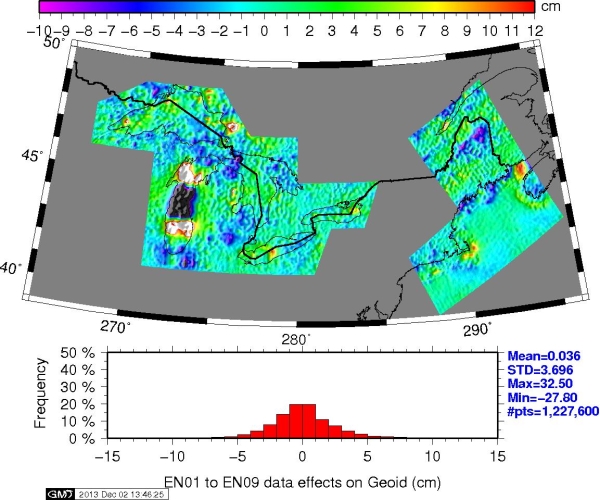
Figure 4 Equivalent residual geoid
signal to that shown in Figure 3.c. Given for GRAV-D aerogravity blocks
over the Northeast U.S. (EN06, EN07, EN09) and Great Lakes (EN01, EN02,
EN03, EN04, EN05).
The process used to develop the residual gravity data
shown in Figure 3c for the Northeast U.S. was repeated for the Great
Lakes region. Figure 4, shows the equivalent residual geoid signal
implied by the residual gravity data for the Northeast U.S. and the
Great Lakes regions. Note that 3-5 mGal features seen in Figure 3c over
Maine translate to 5-10 cm features in the geoid. Systematic differences
in gravity over a hundred kilometers have decimeter impacts on derived
geoid heights. Hence, incorporating the aerogravity would improve the
regional geoid model by modifying surface gravity signal (short
wavelengths) derived from SHM. However, it would be better to not rely
on modifying the suspect surface gravity data after it was incorporated
into a SHM. A better approach would be to remove any biases, trends, or
other systematic effects from the surface gravity data before combining
them into a regional geoid.
The next step after this would be to predict gravity
values from this aerogravity modified SHM at the surface gravity point
locations. However, when this process was developed over other regions
in the Great Lakes, significant ringing occurred. This is normally an
indication of applying too narrow a filter during Spherical Harmonic
Analysis. At the time of this analysis, the issue has not been
adequately resolved to enable a direct comparison using this approach.
An alternative approach was devised that permitted comparison of the
aerogravity signal to existing surface gravity data.
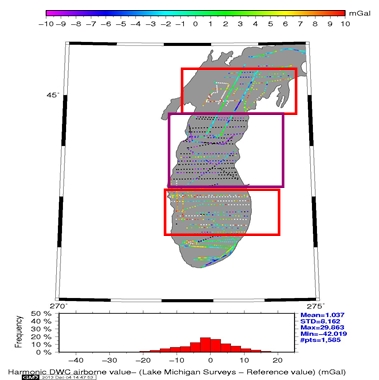
Figure 5 Difference between
aerogravity and surface gravity held in the NGS database. Clear positive
(above +10 mGals) biases are seen in track cluster (red boxes) that
bound a cluster of tracks in the middle (magenta box) where pronounced
negative (below -10 mGals) biases are seen. The scale of these biases
would produce significant systematic errors in derived geoid height
models.
The LSC-generated residual gravity grid was instead
analytically downward continued to the surface. This effectively permits
predictions of the residual values at the locations of surface gravity
survey points. Since the surface gravity have full signal, the original
(GOCO03S-EGM2008) SHM was removed from them to produce a second set of
residuals gravity values. Both sets of residuals have been reduced by
removing the same SHM. Hence, taking the difference of both sets of
residuals highlights the differences between the aerogravity and the
surface data (Figure 5).This double differencing effectively removes the
SHM from the equation, because it is common to both.
Most data fall into an acceptable range of residual
values. Certainly, they will all need to be addressed. However, several
clusters of profiles are seen that have significant systematic effects
(in red and magenta boxes). The cluster of profile lines in the middle
of the Lake are off the bottom of the color scale (black) below -10
mGals in magnitude, while the clusters above and below that are off the
top of the color scale (white) at +10 mGals. Moving from North to South
over these features produces a sharp 20 mGal drop followed by a sharp 20
mGal rise. This feature is clearly seen over Lake Michigan in the same
region in the residual geoid showing signal differences between the
aerogravity and EGM2008 signal in Figure 4. Removal of these biases is
essential if the surface gravity data are to be optimally combined with
the satellite and airborne gravity data into a seamless gravity field
model.
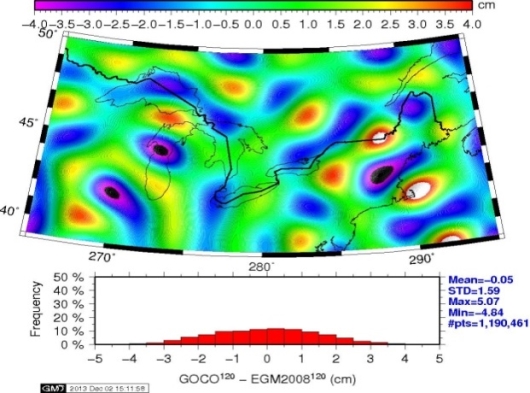
Figure 6 Differences between EGM2008
and GOCO03S through degree 120. Notte that the systematic feature seen
in Figure 5 over central Lake Michigan are seen here but only broadly.
Figure 6 shows the difference between EGM2008 and
GOCO03 filtered to degree 120. This effectively highlights the GOCE
signal in the middle to long wavelengths of the gravity field. Of note,
the same feature is seen over central Lake Michigan as was seen in
Figure 4. Note the same broad structure with a high to the North and
South of a central low. So GOCE would appear to have likewise determined
this same systematic difference with EGM2008. However, the GOCE signal
is too broad to be of use in detecting which specific surface gravity
profiles need to be evaluated for potential biases such as was seen in
Figure 5. While the GOCE-EGM2008 comparison could likely be made as high
as degree 180, this would still be too coarse to enable evaluation of
individual surface gravity profiles as in Figure 5.
4. OUTLOOK AND FUTURE WORK
The National Geodetic Survey will define new
geometric and geophysical datums in 2022 to replace NAD 83 and NAVD 88
for the United States. This paper focused on the aerogravity collected
as a part of the GRAV-D Project to be used for a geoid height model to
serve as the realization of that future vertical datum. Canada has
already adopted a similar datum and Mexico and many other countries in
North and Central America are likewise interested in collaborating on
common geoid models to serve as a regional height system.
Satellite data developed from missions such as GRACE
and GOCE provide the long wavelength control that will unify height
systems both at continental scales and as a part of a World height
System. In turn, the aerogravity data are used to strengthen and enhance
the middle wavelengths for the model over the U.S.A. Surface gravity
data will provide the fine detail at the shortest wavelengths. The aim
is to meld these data sets starting with the satellite data, then
incorporating the aerogravity and finally then using the signal from
surface gravity data - building progressively to a higher resolution
model. The intent is to get away from reliance on existing SHM's
developed using suspect surface gravity data. This will provide a
seamless gravity field model in spectral content as well as spatial
content, because the GRAV D Project will extend well offshore on each
coast and into neighboring countries. At this stage, systematic
differences of between 3-8 mGals still exist in the aerogravity and in
the surface data
Aerogravity processing continues to be refined in an
effort to reduce these effects. The intent is not to rely upon the
satellite data to remove them, but refine the processing techniques such
that that aerogravity agrees with the satellite data in that portion of
the gravity power spectrum where they overlap (transition band). These
updates will result in multiple versions of the data even for the same
block. Expect that the data available on the GRAV-D webpage will be
updated periodically.
Some of the surface gravity data profiles held by NGS
have been demonstrated also to have systematic differences with the
aerogravity. These are likely biases in the surface gravity data whose
source cannot be adequately resolved due to missing metadata. Hence, no
refinements of processing techniques can resolve these. Aerogravity will
be used to detect and mitigate these biases on a survey-by-survey basis.
As the aerogravity processing steps are developed,
procedures for collection and processing will also be refined. These are
already available and serve as basis for contracting some of the
collection work. As these procedures become optimized, they will be
available for others to use to develop standards for collection to be
consistent with global gravity modeling efforts.
Processes for evaluating the surface gravity data
must likewise be developed and improved. Determining an optimal method
for combining data that does not produce ringing either in or out of the
region is essential. Optimally satellite, airborne and surface gravity
must be consistent over their respective transition bands as given in
Figure 1. The aim is to have a seamless gravity field reliant upon
satellite data at the longest wavelengths transitioning through to
aerogravity and finally to surface gravity for the most local control.
It should be noted that the requirement is to define
a geoid model for use as a vertical datum. A more optimal solution would
be to generate a SHM that blends all sources. This would require an
exceptionally large model (degree 10800) to achieve the current
resolution of regional geoid models. This approach would expedite
transformations between various functionals of the gravity field
(gravity anomalies, gravity disturbances, geoid heights, deflections of
the vertical) as well as between height systems (orthometric, normal,
dynamic). It will remain a goal for research to see if this can be
achieved.
There must also be outside metrics to validate the
accuracy of any geoid model derived from this data. The Geoid Slope
Validation Study for 2011 (GSVS11) is documented in Smith et al. (2013b)
and is intended for just this purpose. A new model is planned for later
this year (GSVS14) in a more gravimetrically challenging area (higher
elevations, larger gravity changes though with generally flat terrain).
There will likely be a third GSVS located in the Rocky Mountains to
provide validation in high, rugged terrain.
Additional validation data sets that will be used
include tidal bench marks in combination with Mean Ocean Dynamic
Topography models for validation in coastal regions, astrogeodetic
observations across the region, and minimally constrained GPS on leveled
bench mark (GPSBM) data. The last is a normal step in developing
existing vertical control for NAD 83 and NAVD 88. A minimally
constrained solution is necessary for quality control before a
constrained solution is used to make the official datum values. Minimal
constraints should produce a series of geoid heights representative of
the local geoid. As of 2014, GRAV D is on track for collections and
development of the geoid processing techniques for implementation of a
new vertical datum in 2022.
REFERENCES
Childers VA, RE Bell, and JM Brozena (1999) Airborne
Gravimetry: An Investigation of Filtering, Geophysics, 64 (1), 61-69.
Drinkwater MR, R Haagmans, D Muzi, A Popescu, R
Floberghagen, M Kern, and M Fehringer (2007) Proceedings of 3rd
International GOCE User Workshop, 6-8 November, 2006, Frascati, Italy,
ESA SP-627.
Mayer-Gürr T, D Rieser, E. Hoeck, JM Brockman, W-D
Schuh, I Krasbutter, J Kusche, A Maier, S Krauss, W Hausleitner, O Baur,
A Jaeggi, U Meyer, L Prange, R Pail, T Fecher, and T Gruber. (2012) The
new combined satellite only model GOCO03S. Paper S2-183, GGHS Meeting in
Venice, Italy 9-12 OCT 2012.
NGS (2013) National Geodetic Survey Ten-Year
Strategic Plan,
http://www.ngs.noaa.gov/web/news/Ten_Year_Plan_2013-2023.pdf
Pail R, S Bruinsma, F Migliaccio, C Foerste, H
Goiginger, W-D Schuh, E Höck, M Reguzzoni, JM Brockmann, O Abrikosov, M
Veicherts, T Fecher, R Mayrhofer, I Krasbutter, F Sansò, CC Tscherning
(2011) First GOCE gravity field models derived by three different
approaches, J. Geodesy, 85 (11), 819-843, DOI:
10.1007/s00190-011-0467-x.
Pavlis, NK, SA Holmes, SC Kenyon, and JK Factor
(2012) The development and evaluation of the Earth Gravitational Model
2008 (EGM2008), JGR, 117 (B4), Article Number: B04406, DOI:
10.1029/2011JB008916.
Roman DR (2007) The Impact of Littoral Aerogravity on
Coastal Geoid Heights, paper 9009, XXIV General Assembly of the I.U.G.G.
in Perugia, Italy 2-13 July 2007.
Roman DR, D Winester, J Saleh (2010) Surface gravity
observations define gravity field change over 30 years, Abstract
G41A-0789 presented at 2010 Fall Meeting, AGU, San Francisco, Calif.,
13-17 Dec.
Sandwell DT (1990) Geophysical Applications of
Satellite Altimetry, Reviews of Geophysics Supplement, 132-137.
Saleh J, X Li, YM Wang, DR Roman, DA Smith (2013)
Error analysis of the NGS’ surface gravity database, J. Geodesy, 87:
203¬221.
Smith D (2007) The GRAV-D project: Gravity for the
Redefinition of the American Vertical Datum. Available online at:
http://www.ngs.noaa.gov/GRAV-D/pubs/GRAV-D_v2007_12_19.pdf
Smith DA, M Véronneau, DR Roman, J Huang, YM Wang,
and MG Sideris (2013a) Towards the Unification of the Vertical Datum
Over the North American Continent. Chapter 36 in: Z Altamimi and X
Collilieux (eds.), Reference Frames for Applications in Geosciences,
International Association of Geodesy Symposia 138, DOI
10./1007/978-3-642-32998-2_36 © Springer-Verlag Heidelberg 2013.
Smith DA, SA Holmes, X Li, S Guillaume, YM Wang, B
Bürki, DR Roman, and TM Damiani (2013b) Confirming regional 1 cm
differential geoid accuracy from airborne gravimetry: the Geoid Slope
Validation Survey of 2011, J. Geodesy, 87 (10-12), 885-907.
Snay R and T Soler (2000) Modern Terrestrial
Reference Systems, Part 2: The Evolution of NAD 83, Professional
Surveyor, February.
BIOGRAPHICAL NOTES
Daniel Roman is serving as the Chief (acting),
Spatial Reference Systems Division at the U.S. National Geodetic Survey,
while continuing to serve as the GRAV-D P.I. and Geoid Team Lead for
development of a geoid height model in 2022 that will replace NAVD 88.
Xiaopeng Li is an employee of Data System Technology,
Inc. and is contracted to NGS. He assists with spherical/ellipsoidal
harmonic modeling efforts as well as geoid modeling efforts for various
official and scientific purposes.
ACKNOWLEDGMENTS
The authors wish to thank our colleagues Dr. Simon Holmes, Dr.
Vicki Childers, and Dr. Theresa Damiani for their generous
support in developing this paper.
CONTACTS
Dr. Daniel Roman
National Geodetic Survey
SSMC 3, N/NGS6, #8813
1315 East-West Highway
Silver Spring MD 20910
U.S.A.
Tel. +1-301-713-3200 x103
Fax + 1-301-713-4324
Email: dan.roman@noaa.gov
Web site:
http://www.ngs.noaa.gov/GRAV-D/
http://www.ngs.noaa.gov/GEOID/
 |